We’re familiar with using natural gas every day in heating homes, powering boilers and igniting stove tops. But this same natural gas – predominantly methane – is also one of the most important sources of electricity to the UK. In 2019 gas generation accounted for 39% of Great Britain’s electricity mix. But that could soon be changing.
Hydrogen, the super simple, super light element, can be a zero-carbon emissions source of fuel. While we’re used to seeing it in everyday in water (H2O), as a gas it has been tested as an alternative to methane in homes and as a fuel for vehicles.
Could it also replace natural gas in power stations and help keep the lights on?
The need for a new gas
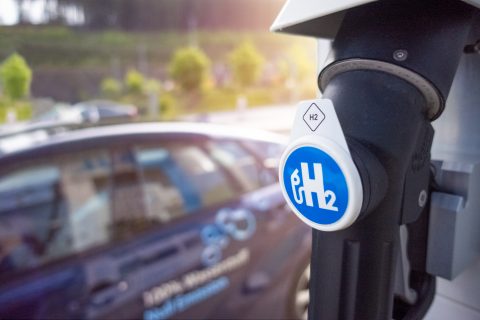
Hydrogen fuel station
Natural gas has been the largest single source of electricity in Great Britain since around 2000 (aside from the period 2012-14 when coal made a resurgence due to high gas prices). The dominance of gas over coal is in part thanks to the abundant supply of it in the North Sea. Along with carbon pricing, domestic supply makes gas much cheaper than coal, and much cleaner, emitting as much as 60% less CO2 than the solid fossil fuel.
Added to this is the ability of gas power stations to start up, change their output and shut down very quickly to meet sudden shifts in electricity demand. This flexibility is helpful to support the growth of weather-dependant renewable sources of power such as wind or solar. The stability gas brings has helped the country decarbonise its power supply rapidly.
Hydrogen, on the other hand, can be an even cleaner fuel as it only releases water vapour and nitrous oxide when combusted in large gas turbines. This means it could offer a low- or zero-carbon, flexible alternative to natural gas that makes use of Great Britain’s existing gas infrastructure. But it’s not as simple as just switching fuels.
Switching gases
Some thermal power stations work by combusting a fuel, such as biomass or coal, in a boiler to generate intense heat that turns water into high-pressure steam which then spins a turbine. Gas turbines, however, are different.
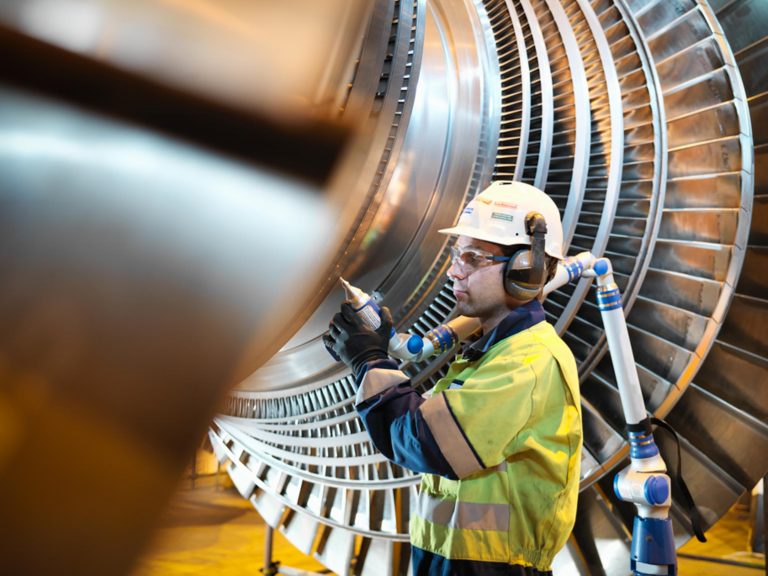
Engineer works on a turbine at Drax Power Station
Instead of heating water into steam, a simple gas turbine blasts a mix of gas, plus air from the surrounding atmosphere, at high pressure into a combustion chamber, where a chemical reaction takes place – oxygen from the air continuously feeding a gas-powered flame. The high-pressure and hot gasses then spin a turbine. The reaction that takes place inside the combustion chamber is dependent on the chemical mix that enters it.
“Natural gas turbines have been tailored and optimised for their working conditions,” explains Richard Armstrong, Drax Lead Engineer.
“Hydrogen is a gas that burns in the same way as natural gas, but it burns at different temperatures, at different speeds and it requires different ratios of oxygen to get the most efficient combustion.”
Switching a power station from natural gas to hydrogen would take significant testing and refining to optimise every aspect of the process and ensure everything is safe. This would no doubt continue over years, subtly developing the engines over time to improve efficiency in a similar way to how natural gas combustion has evolved. But it’s certainly possible.
What may be trickier though is providing the supply of hydrogen necessary to power and balance the country’s electricity system.
Making hydrogen
Hydrogen is the most abundant element in the universe. But it’s very rare to find it on its own. Because it’s so atomically simple, it’s highly reactive and almost always found naturally bonded to other elements.
Water is the prime example: it’s made up of two hydrogen atoms and one oxygen atom, making it H2O. Hydrogen’s tendency to bond with everything means a pure stream of it, as would be needed in a power station, has to be produced rather than extracted from underground like natural gas.
Hydrogen as a gas at standard temperature and pressure is known by the symbol H2.
A power station would also need a lot more hydrogen than natural gas. By volume it would take three times as much hydrogen to produce the same amount of energy as would be needed with natural gas. However, because it is so light the hydrogen would still have a lower mass.
“A very large supply of hydrogen would be needed, which doesn’t exist in the UK at the moment,” says Rachel Grima, Research & Innovation Engineer at Drax. “So, at the same time as converting a power plant to hydrogen, you’d need to build a facility to produce it alongside it.”
One of the most established ways to produce hydrogen is through a process known as steam methane reforming. This applies high temperatures and pressure to natural gas to break down the methane (which makes up the majority of natural gas) into hydrogen and carbon dioxide (CO2).
The obvious problem with the process is it still emits CO2, meaning carbon capture and storage (CCS) systems are needed if it is to be carbon neutral.
“It’s almost like capturing the CO2 from natural gas before its combusted, rather than post-combustion,” explains Grima. “One of the advantages of this is that the CO2 is at a much higher concentration, which makes it much easier to capture than in flue gas when it is diluted with a lot of nitrogen.”
Using natural gas in the process produces what’s known as ‘grey hydrogen’, adding carbon capture to make the process carbon neutral is known as ‘blue hydrogen’ – but there are ways to make it with renewable energy sources too.
Electrolysis is already an established technology, where an electrical current is used to break water down into hydrogen and oxygen. This ‘green hydrogen’ cuts out the CO2 emissions that come from using natural gas. However, like charging an electric vehicle, the process is only carbon-neutral if the electricity powering it comes from zero carbon sources, such as nuclear, wind and solar.
It’s also possible to produce hydrogen from biomass. By putting biomass under high temperatures and adding a limited amount of oxygen (to prevent the biomass combusting) the biomass can be gasified, meaning it is turned into a mix of hydrogen and CO2. By using a sustainable biomass supply chain where forests absorb the equivalent of the CO2 emitted but where some fossil fuels are used within the supply chain, the process becomes low carbon.
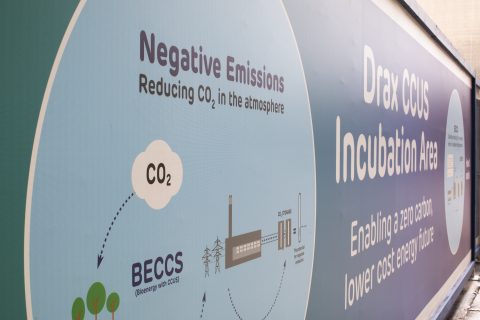
Carbon capture use and storage (CCUS) Incubation Area, Drax Power Station
CCS can then be added to make it carbon negative overall, meaning more CO2 is captured and stored at forest level and in below-ground carbon storage than is emitted throughout its lifecycle. This form of ‘green hydrogen’ is known as bioenergy with carbon capture and storage (BECCS) hydrogen or negative emissions hydrogen.
There are plenty of options for making hydrogen, but doing it at the scale needed for power generation and ensuring it’s an affordable fuel is the real challenge. Then there is the issue of transporting and working with hydrogen.
“The difficulty is less in converting the UK’s gas power stations and turbines themselves. That’s a hurdle but most turbine manufacturers already in the process of developing solutions for this,” says Armstrong.
“The challenge is establishing a stable and consistent supply of hydrogen and the transmission network to get it to site.”
Working with the lightest known element
Today hydrogen is mainly transported by truck as either a gas or cooled down to minus-253 degrees Celsius, at which point it becomes a liquid (LH2). However, there is plenty of infrastructure already in place around the UK that could make transporting hydrogen significantly more efficient.
“The UK has a very advanced and comprehensive gas grid. A conversion to hydrogen would be more economic if you could repurpose the existing gas infrastructure,” says Hannah Steedman, Innovation Engineer at Drax.
“The most feasible way to feed a power station is through pipelines and a lot of work is underway to determine if the current natural gas network could be used for hydrogen.”
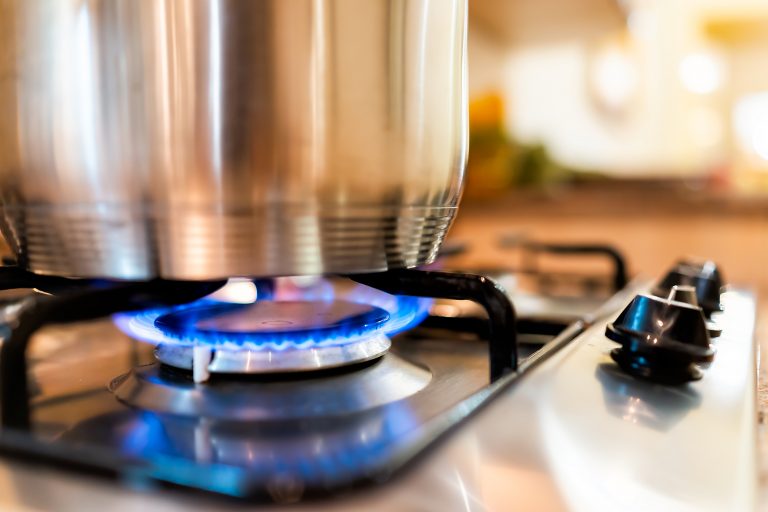
Hydrogen is different to natural gas in that it is a very small and highly reactive molecule, therefore it needs to be treated differently. For example, parts of the existing gas network are made of steel, a metal which hydrogen reacts with, causing what’s known as hydrogen embrittlement, which can lead to cracks and failures that could potentially allow gas to escape. There are also factors around safety and efficiency to consider.
Like natural gas, hydrogen is also odourless, meaning it would need to have an odourant added to it. Experimentation is underway to find out if mercaptan, the odourant added to natural gas to give it a sulphuric smell, is also compatible with hydrogen.
But for all the challenges that might come with switching to hydrogen, there are huge advantages.
The UK’s gas network – both power generation and domestic – must move away from fossil fuels if it is to stop emitting CO2 into the atmosphere, and for the country to reach net zero by 2050. While the process will not be as simple as switching gases, it creates an opportunity to upgrade the UK’s gas infrastructure – for power, in homes and even as a vehicle fuel.
It won’t happen overnight, but hydrogen is a proven energy fuel source. While it may take time to ramp up production to a scale which can meet demand, at a reasonable cost, transitioning to hydrogen is a chance to future-proof the gas systems that contributes so heavily to the UK’s stable power system.